Bursty star formation (SF) and disk settling
Movie: Formation history of a Milky Way-mass galaxy in the FIRE simulation, from Hopkins et al. (2014).
One important prediction from modern cosmological zoom-in simulations that resolve scales below individual star-forming clouds, such as the FIRE simulations, is that SF typically occurs in recurrent bursts in dwarf and high-redshift galaxies, followed by energetic, feedback-driven outflows and gas infall on time-scales ≲ 100 Myr. (e.g. Sparre et al. 2017; Feldmann et al. 2017; Ma et al. 2018a).
Starbursts are not necessarily associated with major/minor mergers. A sharp transition, known as "disk settling", happens when gas forms a rotationally supported disk. Generally speaking, the level of "burstiness" increases with decreasing mass and increasing redshift. Disk settling tends to occur in more massive galaxies at higher redshift. These are in broad agreement with observations.
However, the bursty phase is a poorly understood regime. Independent simulation groups produce severely diverging galaxy properties because of different ISM, star formation, and feedback treatments implemented in these codes (an outstanding example here). The physics driving the burstiness has also been long overlooked.
Disk settling in present-day Milky Way (MW)-mass galaxies
In Ma et al. (2017b, MNRAS, 467, 2430), we investigate the formation history, disk structure, and stellar age and metallicity distribution in a MW-mass disk galaxy by z=0 from the FIRE suite (i.e. the pre-"Latte" simulation). At early times, the galaxy exhibits bursty star formation, with the ISM characterized as highly chaotic and turbulent. Around z~0.8 (i.e. ~7 Gyrs ago), a rotationally supported gasous disk formed, and star formation switched to a time-steady mode in the disk thereafter. The thickness of the gas disk decreased with time due to decreasing gas fraction (see the left panel in the figure below).
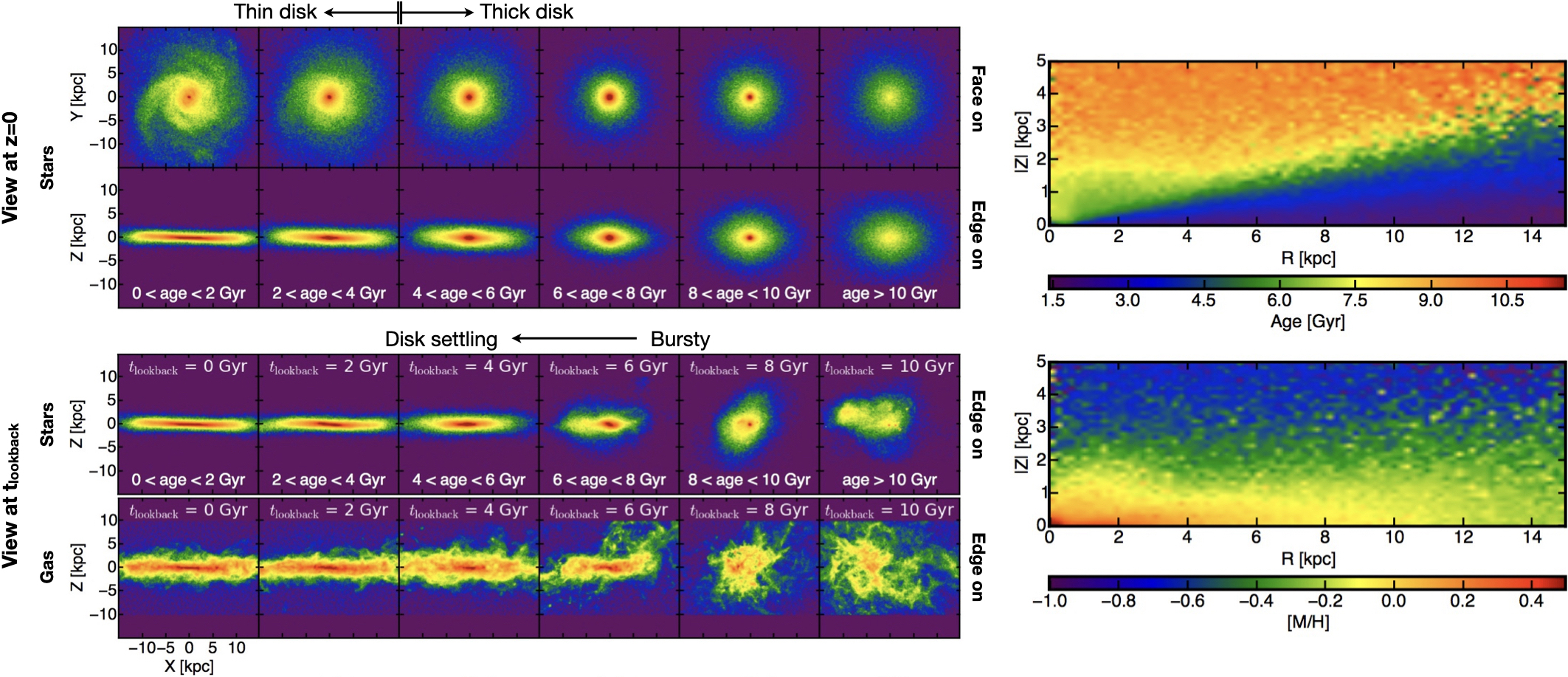
Figure: Left: Disk settling process in a MW-mass galaxy at z=0 from the FIRE simulations (bottom). The bursty and time-steady phases leave different imprints in the stellar morphology at the present day (top). Right: The median stellar age (top) and metallicity (bottom) in the galactic disk, in broad agreement with what observed in our MW. This is naturally reproduced by the bursty-to-steady formation history of this galaxy. Taken from Ma et al. (2017b).
We found that such a formation history can naturally reproduce the observed two-component vertical disk structure in our MW, known as the thin disk and the thick disk. It also results in the stellar age and metallicity distribution in the stellar disk (see the right panel in the figure above), with radial and vertical stellar metallicity gradients in good agreement with what observed in the MW. In a companion paper (Ma et al. 2017a), we present the gas-phase metallicity gradient for this galaxy (see more details here).
What processes drive the starburst?
Movie: A starburst cycle in a M*~ 109M⊙ galaxy at z~5, from Ma et al. (2020a).
The movie on the left presents a starburst episode in a z ~ 5 galaxy with Mhalo~ 1011M⊙ and M*~ 109M⊙. It shows four stages of a starburst:
(1) Gas falls back from the last burst, building up a gas-rich, turbulent environment in the ISM.
(2) Star formation begins. Supernova feedback blows out superbubbles, which compress the dense gas nearby and trigger new star formation at the bubble front as they sweep up the ISM.
(3) By this means, star formation propagates in the ISM. Superbubbles blown out around each star formation site overlap.
(4) Almost all gas is evaculated in the ISM as outflows. The gas will fall back and triggers the next starburst as stage (1).
The key process here is that feedback (mainly supervovae) can compress the dense gas and trigger star formation nearby, such that the gas is severely disturbed before circularized. We demonstrate that this process is crucial for the formation of globular clusters in high-redshift galaxies (Ma et al. 2020a; see details here) as well as the escape of Lyman-continuum photons for cosmic reionization (Ma et al. 2020b; see details here).
However, we need to be cautious as our simulations cannot fully resolve the structure of radiative shocks at the front of the superbubbles nor include a chemical network for dust and H2 molecules to model star formation more accurately, so we may not produce the correct amount of stars formed in the compressed shell or the exact time-scale for star formation to occur there. We plan to investigate this in more detail using ultra-high-resolution of small patches of the ISM with self-consistent radiation-hydrodynamics and molecular networks.
How do rotationally supported disks form?
Collaborators and I have found that present-day ~L* galaxies form rotationally supported disks around z ~ 1 in the FIRE simulations (Ma et al. 2017a; Garrison-Kimmel et al. 2018). The characteristic stellar mass for disk formation increases with redshift. This trend is broadly consistent with the observed fact that disk fraction decreases with redshift at fixed stellar mass (e.g. Kassin et al. 2012; Wisnioski et al. 2015, 2019), but still, FIRE is likely too difficult to produce disks.
In Stern et al. (2020), we report that disk settling in the FIRE simulations coincides with the virialization of the inner circumgalactic medium (CGM) (i.e. when a hot inner halo forms). The causality is not clear, although we speculate this is because (a) gas can circularize before cooling efficiently and forming stars due to the long cooling time and (b) the hot halo confines outflows, preventing them from damaginig the galaxy.
I recently start a numerical experiment as follows. Taking the gas distribution prior to a starburst from my FIRE cosmological zoom-in simulations and putting it in an isolated halo of similar mass and concentration, I manually add a stellar bulge with varying mass and scale length. Interesting enough, I find as long as the bulge is sufficiently massive or compact, a gas disk can form (see figure below). This suggests that a deep gravitational potential can prevent feedback from disrupting disk settling process.
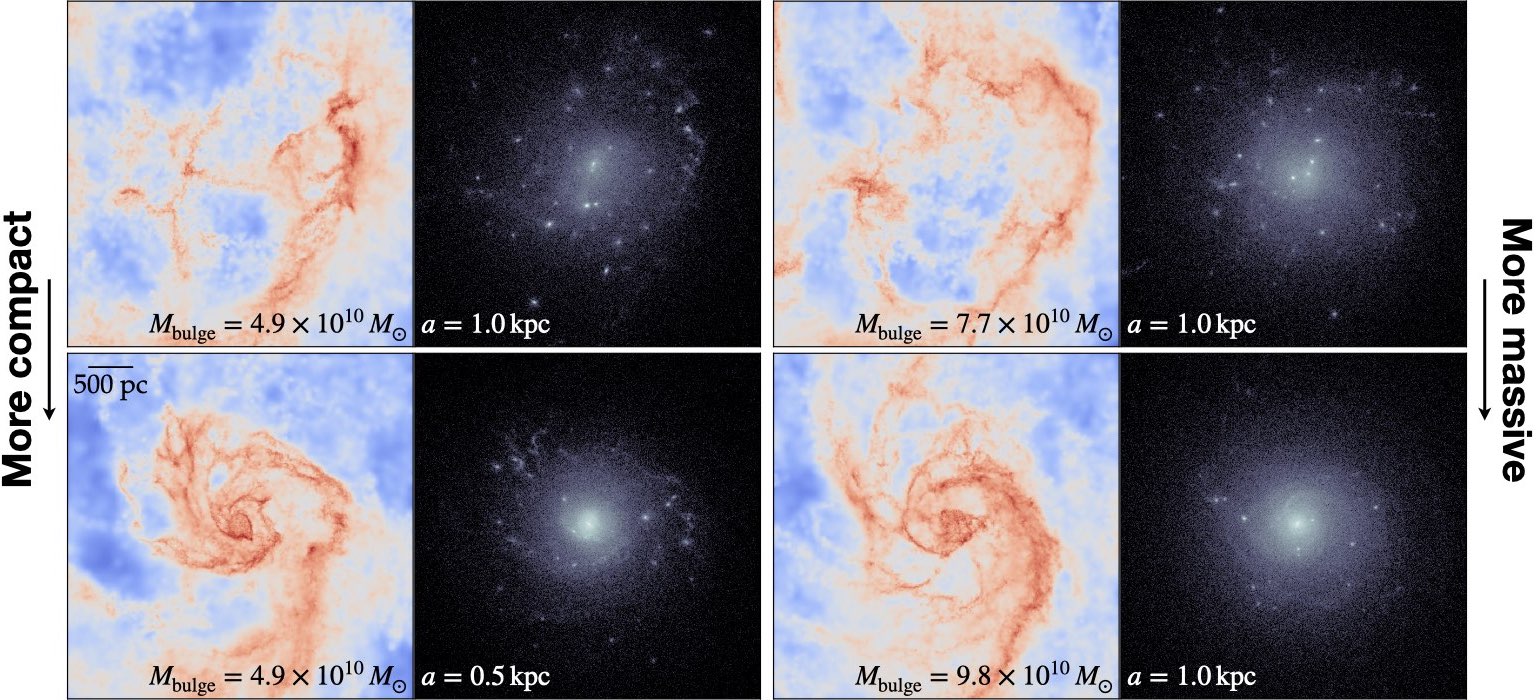
Figure: An idealized disk settling experiment in a Mhalo~ 1012M⊙ halo at z ~ 8. It suggests that a sufficiently massive or compact stellar bulge can prevent feedback from destroying disk settling process (work in progress).
Back to research