Overview
My research interests are broad, and nearly any topic related to stars or planets is fascinating to me. Although I'm a theorist, my group tends to work on problems related to observational puzzles. Much of our work focuses on fluid dynamics within stars and planets, and our methods include a variety of analytical and numerical calculations coupled with sophisticated stellar evolution codes and hydrodyamics simulations. You can learn more about our research below.
Mass Transfer
Many stars reside in binary systems in which one star (or both) will expand and transfer mass to the other. The mass transfer phase is crucial for producing many types of unusual stars, the formation of gravitational wave sources such as binary black holes, and the appearance of various types of supernovae. However, we do not understand if the mass transfer can remain stable, and how the orbit of the stars evolves in response to the mass transfer. Our group has been performing hydrodynamic simulations of stellar mass transfer to determine the mass and angular momentum loss that occurs during this phase, in order to understand the long-term evolution of such systems.
Red Supergiant Mass Loss
Stars more than about 10 times the mass of the Sun evolve into red supergiants at the end of their lives. It is well known that these stars progressively blow off their extended outer envelopes as they evolve, but the physics driving their mass loss is not well understood. My group has investigated this topic by examining the shock waves driven by convective motions near the surface of the star, finding that these shock waves drive material into an extended chromosphere surrounding the star. Any material extending more than a few times larger than the surface radius can cool off enough to allow dust to form. The mixture of gas and dust is then blown away by the pressure of photons emitted by these very luminous stars. We find that the star's chromosphere also has a huge effect on the appearance of supernovae produced by these stars in the first few days after they explode, an effect which has been observed in many recently discovered supernovae.
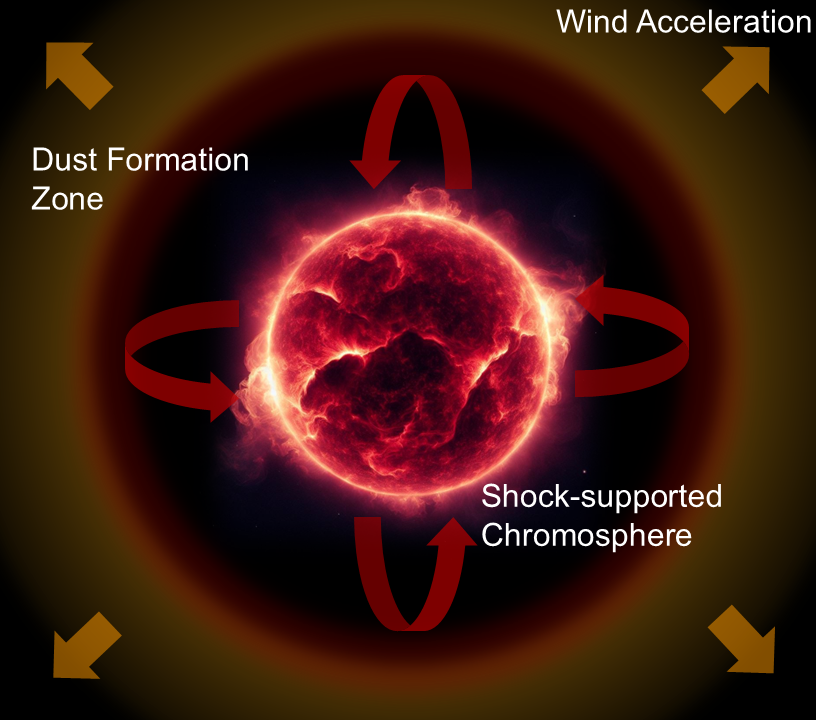
Here is our article on the subject:
Saturn Ring Seismology
Seismology is our most powerful tool for understanding the interiors of stars and planets. Indeed, our knowledge of the interior structures of the Earth and Sun owes its existence primarily to seismic measurements. Unfortunately, remotely detecting seismic disturbances on planets other than the Earth is very difficult, and until recently such a feat had never been achieved. Intriguingly, Saturn offers an unprecedented opportunity to observe seismic oscillations through their gravitational interactions with Saturn's rings. Recent observations of Saturn's ring system have revealed the presence of density waves within the rings excited by global oscillation modes of Saturn. Hedman & Nicholson (2013) used observations of Saturn's rings with the Cassini satellite to measure the frequencies of the waves in the rings, thereby precisely measuring a small set of the planet's mode frequencies. These observations reveal the presence of unexpected planetary oscillation modes, finely split in frequency from those expected, and whose presence may shed new light on Saturn's interior structure.
Above: Animation of an oscillation mode of Saturn creating waves in the rings. Credit: Robert Hurt
Below: Cartoon of the interior structure of Saturn inferred from my seismic analysis.
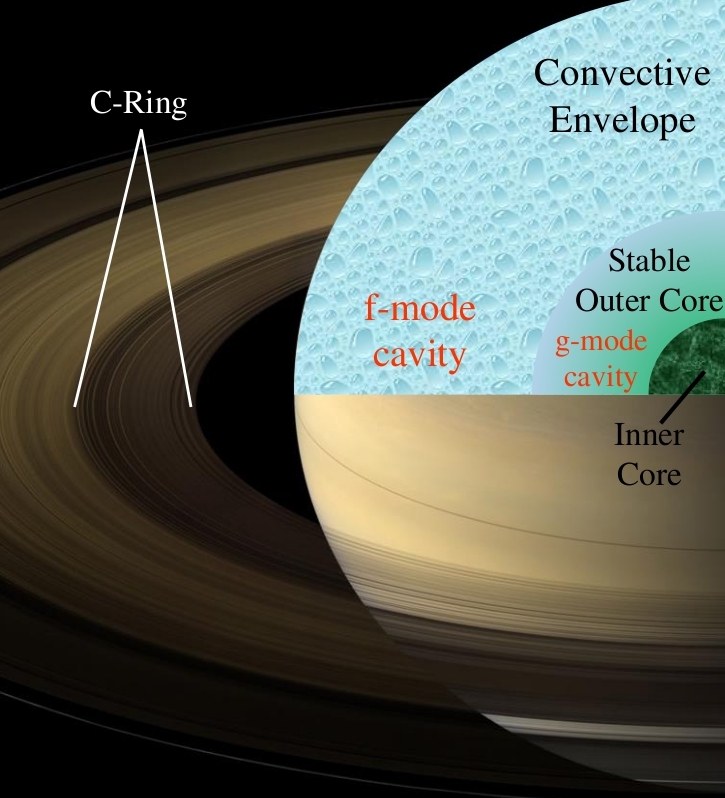
My group's work has focused on the origin of the unexpected oscillation modes of Saturn, and to understand their implications for its structure. Along with postdocs Chris Mankovich and Janosz Dewberry, we have constructed planetary models and computed the corresponding mode frequencies, comparing them with the observed mode frequencies. The frequencies of the modeled fundamental modes match the observed frequencies to an accuracy of roughly 1%. The additional unexpected modes only exist in models containing a large stably stratified (non-convective) planetary core. The stable stratification allows for the presence of gravity modes which can mix with the fundamental modes to obtain a detectable influence on the rings. Saturn's core must be diffuse or "fuzzy", containing a gradual transition from a gaseous envelope to a icy/rocky core.
Here are our articles on the subject:
Triaxial Pulsations
When stars are in binary systems, the gravity of their companion star distorts the star away from its spherical shape, into an elliptical shape known as a triaxial ellipsoid. The distorted shape of the star acts as a lense that diffracts sounds waves propagating in their interiors. This means that any pulsations of the star have a different geometry than those of a spherical star. Our work computed this geometry using perturbation theory, for stars that are mildly tidally distorted. Unlike normal stellar oscillations that have spherical harmonic patterns aligned with the stars rotation axis, the pulsations of tidally distorted stars become aligned with the principal axes of the star and have a fixed pattern relative to the companion star. This modulates the observed amplitudes and phases of the star's pulsations in a very characteristic pattern that has now been observed in dozens of stars. The movies below show some examples of pulsation geometries as the companion star orbits the pulsating star.
We recently submitted an article on this subject which will be available soon!
Pre-Supernova Outbursts
Massive stars explode as supernovae when they die, but historically they were not predicted to give any warning signs that they are about to explode. However, observations of many types supernovae now show evidence for mass loss or eruptions from the star in its final years of life. In a few cases, these outbursts have been observed directly. These eruptions may be linked to evolution in the core of the star, where the nuclear energy generation rate increases by a factor of roughly one million over the last decades of the star's life. However, most of this nuclear energy escapes in neutrinos, and photons in the core of the star cannot diffuse to the surface before the star explodes, so another form of energy transport would be needed to link nuclear energy generation in the core with an outburst at the surface of the star.
My group has examined the role of energy transport by hydrodynamic waves that are excited as a byproduct of the core convection associated with energetic nuclear burning. Our work shows these waves can carry a small fraction of the nuclear energy toward the surface of the star, and that waves can become the dominant form of energy transport in the last years of a star's life. The waves damp and deposit heat in the envelope of the star, which can cause the star to become much brighter at its surface and generate a pre-supernova outburst. The density structure of the star may also be affected, which will alter the appearance of the subsequent supernova.
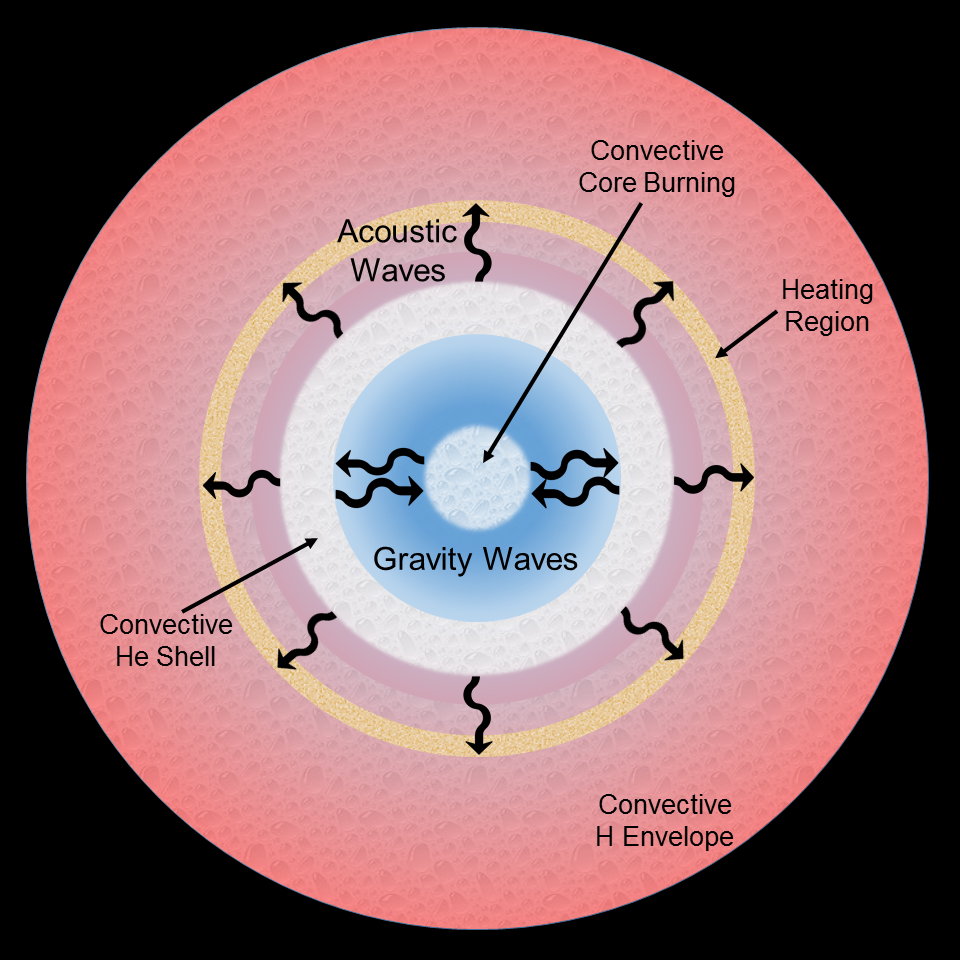
Above: Cartoon picture showing how waves excited in the core of a star can travel into the envelope of a star, where they deposit enough energy to power an eruption from the surface of the star.
Here are our recent articles on the subject:
We have also found that extreme pre-supernova mass loss can occur even in the absence of energy transfer by waves. In low-mass helium star supernova progenitors, the star expands suddenly in the last decade of its life due to intense helium shell burning. When such stars are in binary systems, this can drive enormous mass loss rates that may help explain the emerging classes of type Ibn supernovae and ultra-stripped supernovae.
Tidal Migration of Moons and Exoplanets
Just as our own moon is slowly moving away from the Earth, the moons of Jupiter and Saturn have slowly migrated outward over the history of the solar system. Recent measurements from have revealed that Saturn's moons are currently migrating much faster than astronomers had guessed, including its largest moon Titan. The rapid migration leads to tidal heating of the moons and helps explain why Saturn's moon Enceladus is volcanically active.
Here is the discovery of the outward migration of Titan:
My research has attempted to explain the rapid migration (and predicted it for Titan) through a process called resonance locking. In this scenario, moons get trapped in resonances with oscillation modes of their host planets. As the planets evolve, the resonant semi-major axes associated with the oscillation modes can move away from the planet, carrying moons with them. The migration of the moons is therefore determined by the thermal evolution of the planet, which proceeds on a timescale shorter than conventional ("constant Q") tidal migration, and hence the moons migrate faster than expected.
Above: Animation showing how a moon can "surf" a resonance lock outward.
Here is the paper predicting outward migration of Titan:
Click below for a cool movie describing some related work:
Just as planetary moons can migrate via resonance locking, so can exoplanets that are close to their host stars. In this case, the exoplanets usually orbit faster than the star rotates, causing the planet's orbit to decay at about the same rate that the star evolves. This can cause the orbital evolution to be much faster (or slower) than predicted by other theories, and has a unique imprint on the distribution of exoplanet orbital periods.
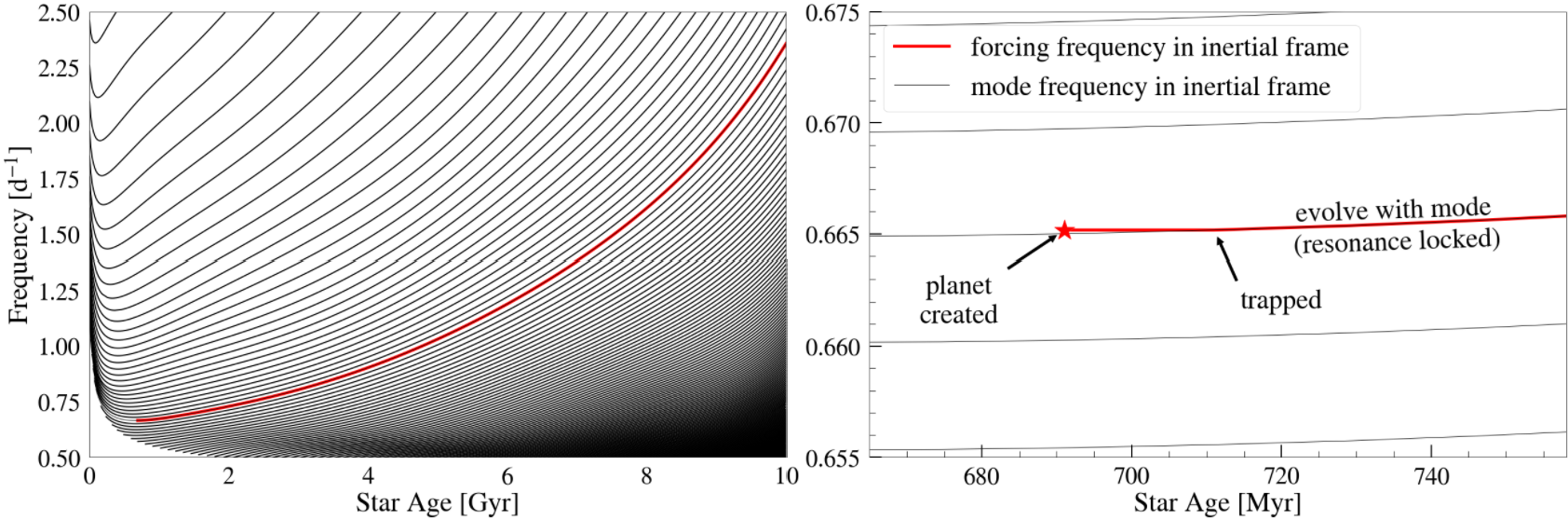
Above: As a star evolves, its oscillation mode frequencies increase with time, sweeping up nearby planets and causing them to migrate inwards, toward their impending doom.
Here are our recent articles on the subject:
Uncovering Stellar Mergers with Asteroseismology
About one out of every ten stars merges with another star during its lifetime. However, identifying stars that have already merged with a companion can be difficult if you don't know what to look for. My group has bee predicting the affect on the structure of the star, and how this imprints the fingerprint of a prior merger in the asteroseismic pulsation spectrum of a red giant star. By looking for this fingerprint amongst asteroseismic catalogs, we have identified a couple dozen red giant stars that likely underwent a merger in their recent past.
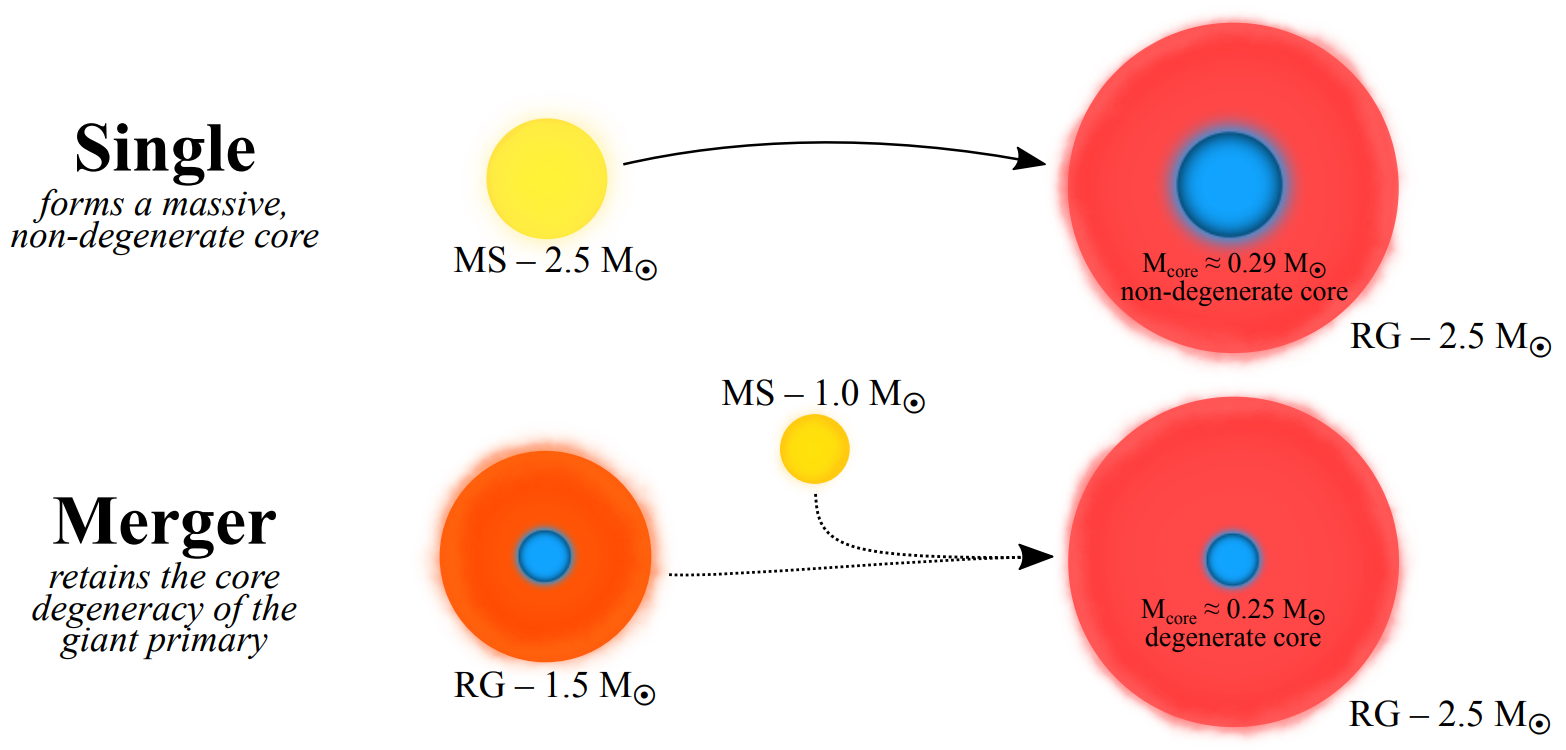
Above: Stars that merge on the red giant branch have dense, degenerate, undermassive cores compared to single stars or stars that merge on the main sequence. This means their asteroseismic period spacing is smaller than expected for their mass, allowing them to be identified.
Here is our recent article on the subject:
Merging White Dwarfs
White dwarfs with short orbital periods emit gravitational waves, which causes the orbit to decay until the white dwarfs merge or begin mass transfer. Much of my graduate work focused on the tidal interactions in the white dwarfs before they merge, which effect the outcome of the merger or mass transfer process. Along with Kevin Burdge and Tom Prince, we are now finding these binary white dwarfs with ZTF, allowing for a new understanding of the population of merging white dwarfs. The movie above depicts the recently discovered system J1539+5027, the shortest period eclipsing binary star known, with an orbital period just under seven minutes.
Movie made by Robert Hurt.Here are a couple recently discovered white dwarf binary systems:
The image below shows my inner artist's conception of the J0651 binary white dwarf system, which has an orbital period of just 12.75 minutes. The sizes/separations of all objects below are shown to scale! These systems are placing new constraints on tidal spin-up and heating of the white dwarfs, in addition to the physics of binary stars that gives rise to these systems.
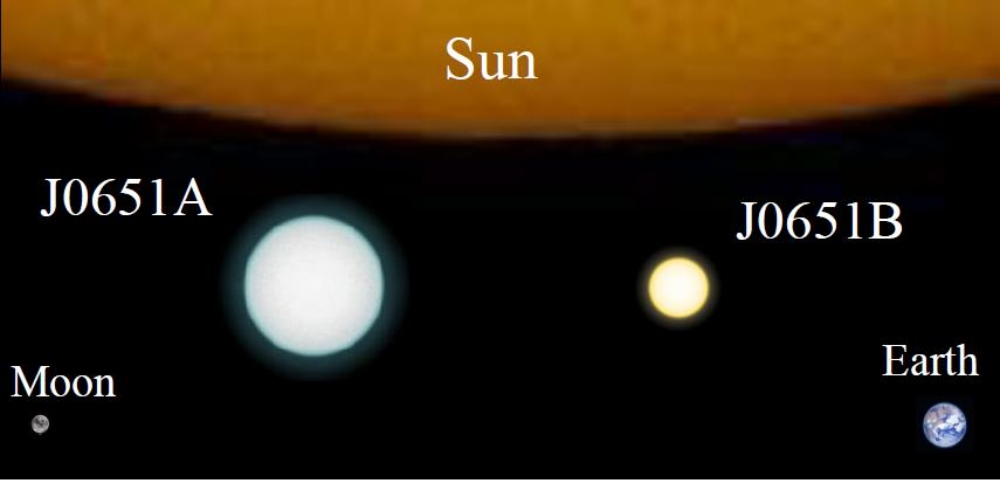
Compact Object Rotation
Above: Animation of a differentially rotating star, where inner layers rotate faster than the outer layers. This shear creates instabilities that act like friction and bring the star closer to a rigidly rotating configuration.
The rotation rates of compact objects (such as white dwarfs, neutron stars, and black holes) are determined by the angular momentum inherited from the progenitor star. It is the rotation rates of the cores of these stars (which are difficult to measure) that are most important, but these rotation rates are determined by uncertain processes that redistribute angular momentum within stars as they evolve. Fortunately, asteroseismic observations have recently directly measured the core rotation rates of many stars, allowing us to develop a better theoretical understanding of the physical mechanisms at work.
Along with collaborators Tony Piro, Adam Jermyn, and Linhao Ma, we have investigated angular momentum transport due to magnetic torques caused by the Tayler instability. We have found that these torques predict internal rotation rates roughly consistent with those measured by asteroseismology. We are now making predictions for the spins of neutron stars and black holes, finding that these compact objects may be born spinning much slower than prior expectations.
Here are my group's recent articles on the subject:
Another possibility is that neutron stars have rotation rates determined by the chaotic processes occurring in the final months of its parent massive star's life. During this period, nuclear burning proceeds so rapidly that the star's core is irradiated by waves launched by extremely vigorous convection. These waves are powerful enough to change the spin direction and spin rate of the core, such that its spin undergoes a random walk. The spin period of the core at the end of the random walk (when the supernova occurs) is tantalizingly similar to that needed to match the observed spin periods of young pulsars!
Below: Animation of the spin-up of a massive stellar core (here made to look like the Earth). The direction of the rotation axis varies randomly, although on average the spin frequency slowly increases in time.
My article on the subject:
Magneto-asteroseismology
Magnetic fields are an important but poorly understood aspect of stellar evolution. In the past, our inability to measure magnetic fields within stars has hampered the development of a coherent picture of stellar magnetism. Now, asteroseismology is offering the first glimpses of magnetic fields within stars. In red giant stars, pulsations at the surface of the star are created by waves that travel from the surface of the star, into its core, and back to the surface.
Above: Animation showing the ray path of a wave propagating in a red giant.
Below: Cartoon illustrating the trapping of a wave within a red giant with a magnetized core, referred to as the magnetic greenhouse effect.
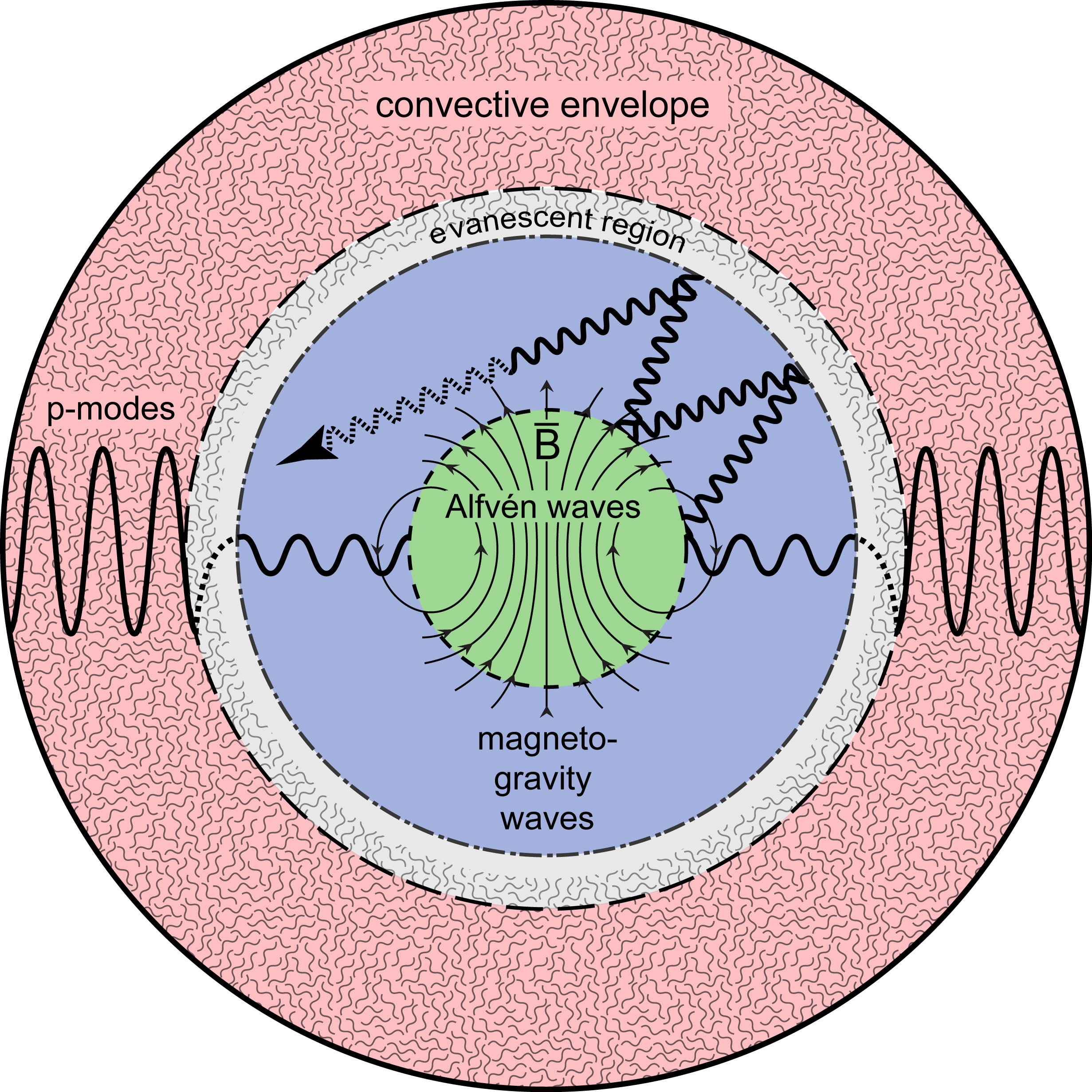
If a strong magnetic field lurks within the star's core, the picture changes. When the wave encounters the strong magnetic field, it is redirected by magnetic tension forces. This changes the nature of the wave, such that it can no longer escape from the star's core and propagate back to the surface. The loss of the wave energy at the surface of the star results in a decreased stellar pulsation amplitude, signaling the presence of a strong magnetic field in the star's core. This effect is observed in roughly one out of five red giants, whose cores contain magnetic fields thousands to millions of times stronger than typical refrigerator magnets!
Our papers can be found at
Heartbeat Stars
Heartbeat stars are an emerging class of eccentric short-period binary stars. The characteristic "heartbeat'' signal evident in their light curves is produced by a combination of tidal distortion, reflection, and Doppler boosting near orbital periastron. Many heartbeat stars continue to oscillate after periastron, indicative of the tidal excitation of oscillation modes within one or both stars. These systems are among the most eccentric short period binaries known, and they constitute an exciting opportunity to observe and understand tidal effects in action.
The video above shows an animation of the orbit of the heartbeat star KOI-54. The bottom panel shows the light curve, which contains a spike near periastron (due to the effects listed above) and oscillations away from periastron due to tidally excited stellar oscillations modes. The stellar oscillations cuase the stars to dissipate orbital energy, causing their orbit to slowly circularize. My research seeks to understand the tidally excited oscillations and their effect on the orbital evolution of eccentric binary stars.
What would a heartbeat star sound like? Click below to find out! To generate this "sound curve", I have converted the light curve (measured by Kepler) into a sound byte, and sped it up by a factor of 5 million. The "heartbeat" sound you'll hear is produced by the tidal distortion, reflection, and Doppler boosting at periastron. The steady tone is produced by the tidally excited oscillations.
Here are our articles on the subject: